Astronomical observations over the past 60+ years have revealed the presence of over 270 molecules outside the Solar System. They are found in environments related to nearly all aspects of stellar evolution: cold interstellar clouds, star-forming regions, protoplanetary disks, late/evolved stars, and in the remnants of planetary nebulae. To understand how these molecules are formed, what other molecules may be present, and what this chemistry tells us about the origins of life on Earth (not to mention the prospects for life elsewhere in the universe), astrochemists rely on chemical models which attempt to simulate the chemistry as a function of time and physical conditions. These models simulate reactions happening between molecules in the gas phase, on dust surfaces, and in interstellar ices, leading to predictions of molecular abundances as a function of time (and sometimes as a function of position within an object).
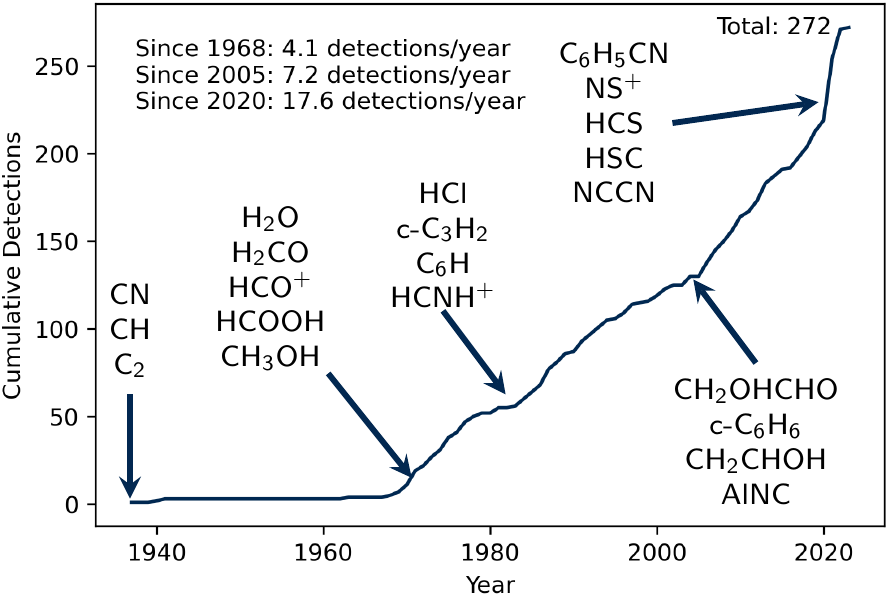
In the 2010s, the surprising discovery of so-called “Complex Organic Molecules” (COMs)– carbon-bearing molecules containing 5 or more atoms, such as methanol (CH3OH)– in prestellar cores upended much of the conventional wisdom in the field. Prior to this discovery, astrochemical models had found that COMs could only be produced in reactions occurring within cold interstellar ices, where they would remain trapped until a newly-born protostar began to heat and evaporate the ices. A prestellar core, however, by definition does not have a protostar! Prestellar cores are some of the coldest objects in space (<10 K), well below the temperature needed to desorb COMs from ices. So how are these molecules being formed, and if they are present, what other chemistry can they do? While many hypotheses are being explored around the world, our group is focused on the role that gas-phase reactions may play in explaining cold COM astrochemistry.
At such low temperatures, chemistry is hard. Most chemical reactions possess activation barriers, and at 10 K, essentially any activation barrier will render a chemical reaction impossible: there is just not enough energy to surmount the barrier. But there are two types of reactions that get around this problem: reactions without an activation barrier (usually involving an exotic molecule such as an ion or a radical), and reactions that have a small barrier but can proceed by quantum mechanical tunneling through the barrier. Both of these types of reactions present an extraordinary challenge for theoretical calculations and for experimental measurements, and as a result, only a small fraction of the reactions in astrochemical models have actually been investigated at astrophysically relevant temperatures. Moreover, the models themselves may be incomplete– missing certain reactions which may well be important in space. Our group is interested in providing experimental measurements of reactions involving known astronomical radicals (e.g., OH, SH) and COMs that possess H-bonding functional groups.
To do this, we seek to use the CRESU technique combined with chirped-pulse Fourier transform microwave (CP-FTMW) spectroscopy. CRESU is a French acronym which means “reaction kinetics in a uniform supersonic flow,” and it has been used since the early 1980s to measure radical reactions at temperatures as low as 7 K. In CRESU, a sample gas containing reactants of interest is flowed into a vacuum chamber through a converging-diverging de Laval nozzle, which is carefully designed to cool the gas to a particular target temperature and generate a molecular beam which is uniform in temperature and density. A pulsed laser is then used to generate a radical of interest, and the abundance of that radical as a function of time is traditionally monitored with laser-induced fluorescence spectroscopy. We are combining the CRESU approach with the powerful CP-FTMW spectroscopy technique to provide real-time measurement of the molecular beam composition, providing new insights into the kinetics and dynamics of the reactions occurring in the flow.
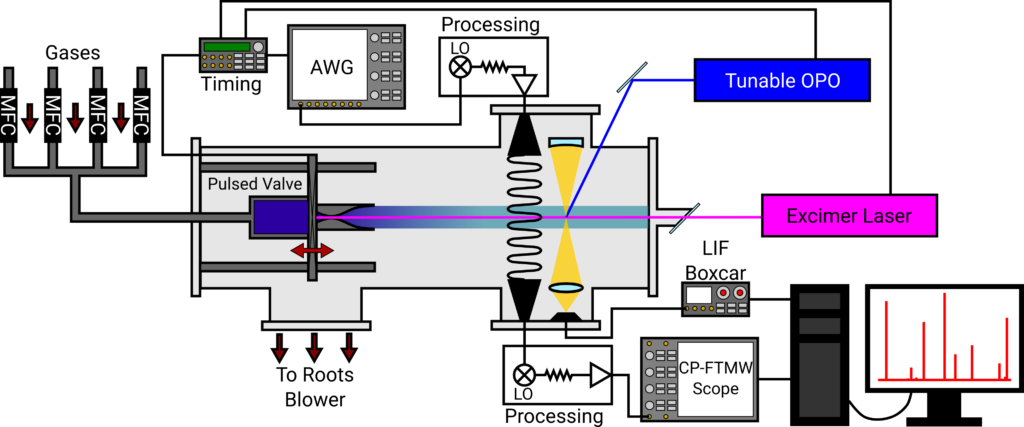
However, one major challenge for coupling these two techniques exists: the density in the uniform molecular beam is too high. CP-FTMW spectroscopy requires that the molecules be able to freely rotate for a few microseconds, but molecules in the uniform flow experience collisions every ~100 nanoseconds or so. Some groups have attempted to get around this by introducing a skimmer to extract the core of the flow into a differentially pumped vacuum chamber to decrease the density. But this can introduce shocks into the flow, disrupting the critical uniformity needed for accurate reaction rate measurements.
We are employing an approach developed by Arthur Suits (University of Missouri) involving the use of an extended Laval nozzle. In this approach, the reaction occurs within an extension attached to the end of a traditional Laval nozzle, then the gas is allowed to expand into a high vacuum chamber. This “freezes” the chemistry by rapidly decreasing the density and temperature, creating a more ideal environment for CP-FTMW spectroscopy.
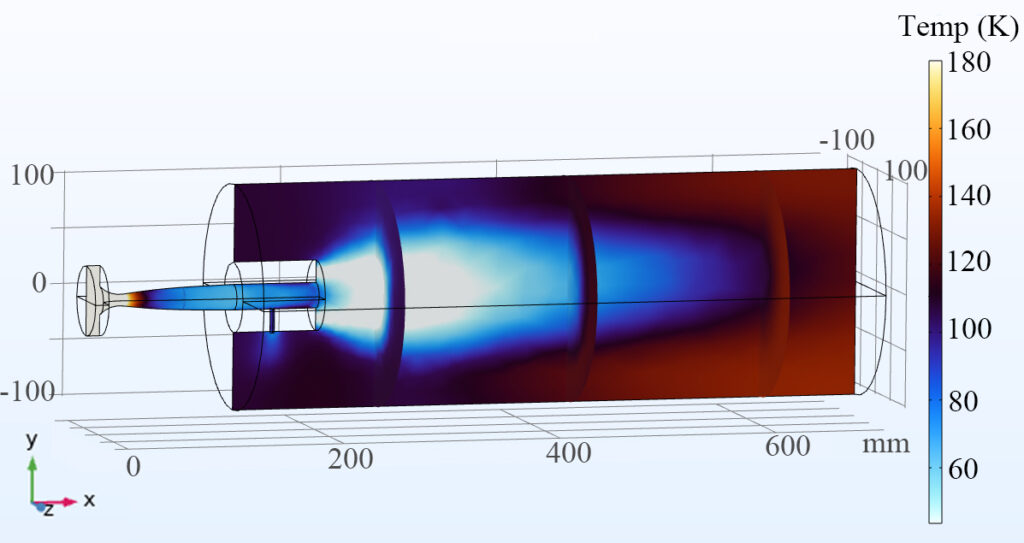
We have adapted this idea to include an optical element into the extended flow, enabling the use of laser-induced fluorescence (LIF) spectroscopy inside the uniform region. This allows us to measure the rate of reactant loss directly in the uniform flow to complement the product detection in the secondary expansion. Using impact pressure measurements and computational fluid dynamics simulations, we have shown (below) that adding a hole into the nozzle extension produces a shock wave in the flow which is eliminated when we fill the hole with an optical element.
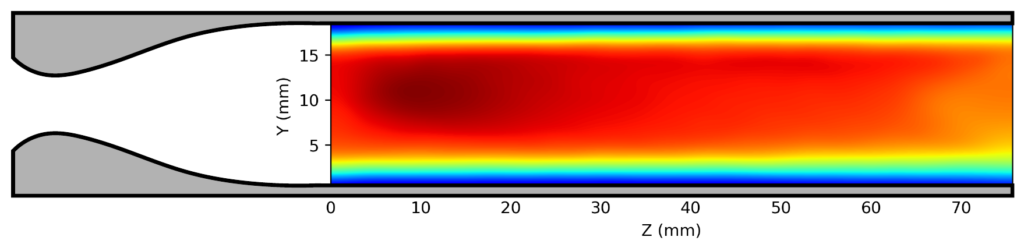
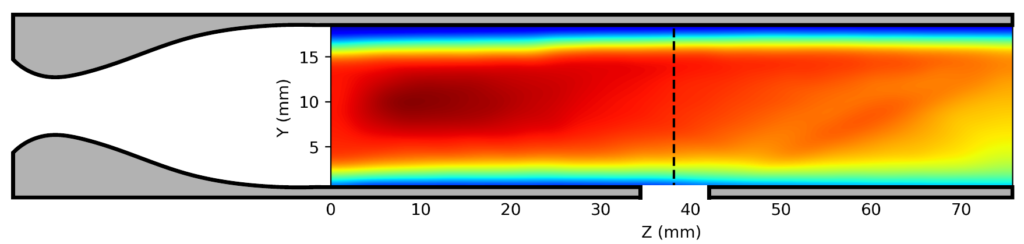
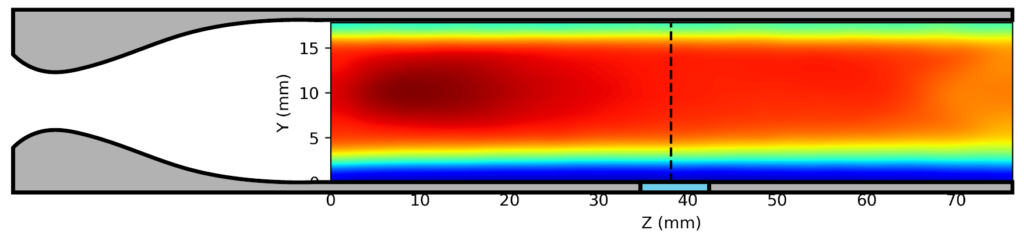
(top) Standard extension
(middle) 4mm diameter hole
(bottom) 4mm hole plugged with an optical window
Our ongoing work is to expand the range of Laval nozzles and extensions we have to generate a range of temperature and density conditions for kinetics, and to perform kinetics experiments for reactions involving the OH and SH radicals.